About the topic
Bio
Get the Flyer (pdf)
About the topic
Eric writes: Tiny particles called neutrinos are one of the fundamental building blocks of our universe. Enormous numbers of neutrinos are produced inside stars and supernova explosions, and they are so numerous that trillions of them have harmlessly passed through you in the time it has taken you to read this sentence. With no electric charge and practically no mass, neutrinos can travel through light-years of rock or lead without being disturbed. Recent observations that neutrinos can change from one type to another type have shown that, surprisingly, the neutrino's mass is not zero. This fact opens up the possibility that neutrinos played a critical role in the development of matter and antimatter in the universe after the Big Bang. Many experiments are now seeking to uncover the properties of neutrinos, including the Tokai to Kamioka ("T2K") project in Japan. We are using a powerful particle accelerator to create a beam of neutrinos, and send them through the earth to the Super-Kamiokande neutrino detector on the other side of the country. I'll explain why we're doing this, how we make neutrinos, and how we detect them.
Super-Kamiokande (Super-Kamioka Nucleon Decay Experiment) is a large water Cerenkov detector. The construction was started in 1991 and the observation began on April 1, 1996. The Super-Kamiokande is operated by an international collaboration of about 110 people and 30 institutes from Japan, the United States, Korea, China, Poland and Spain. The Super-Kamiokande detector consists of a stainless steel tank, 39m diameter and 42m tall, filled with 50,000 tons of ultra pure water. About 13,000 photo-multipliers are installed on the tank wall. The detector is located at 1000 meters underground in the Kamioka mine, Hida-city, Gifu, Japan. The first Kamiokande made the news on 12 November 2001 when a photomultiplier exploded, starting a shockwave in the tank that took out about 6,600 others. By 2006 the damage was repaired and the machine upgraded; it's now Super-K III.
A neutrino (they are denoted by ν, the Greek letter nu) interaction with the electrons or nuclei of water can produce a charged particle that moves faster than the speed of light. This creates a cone of light known as Cerenkov radiation, which is the optical equivalent to a sonic boom. The Cerenkov light is projected as a ring on the wall of the detector and recorded by the PMTs. Using the timing and charge information recorded by each PMT, the interaction vertex, ring direction and flavor of the incoming neutrino is determined. Neutrinos come in electron, muon, and tau flavors, and each has its corresponding antineutrino.
From David Casper, University of California Irvine: In five distinct measurements, Super-Kamiokande finds neutrinos apparently "disappearing". Since it is unlikely that momentum and energy are actually vanishing from the universe, a more plausible explanation is that the types of neutrinos we can detect are changing into types we cannot detect. This phenomenon is known as neutrino oscillation. Neutrino oscillation is not black magic - there are very specific predictions for the behavior of our data if neutrinos oscillate, and we have uniformly found the data in good agreement with these predictions. Unfortunately, a non-mathematical explanation of why neutrino oscillation and neutrino mass are inseparable is difficult. A more detailed, yet still non-mathematical explanation, and a full calculation, using basic quantum mechanics, are provided for those inquiring minds who want to know.
It is much easier to explain why our data appear to lead inexorably to the conclusion that neutrinos are oscillating. The secret lies in the fact that the neutrinos observed by Super-Kamiokande are without exception produced at great distances from the detector. Neutrinos produced in the atmosphere arrive at the detector from distances of about 40 km (if produced above it) to 12,000 km (if produced on the other side of the Earth). These distances are significantly greater than any measurements made to date with neutrinos from accelerators or nuclear reactors on Earth. The Sun, clearly, is much, much further still. The great distances not only allow us to detect effects which would be invisible with a closer neutrino source, they also allow us to measure the behavior of neutrinos produced over a great range of distances. This ability in fact leads to some of the most dramatic evidence that oscillations are occurring.
The probability of a neutrino changing type is related to the distance travelled by the neutrino from its point of production to its point of detection. As a general rule, neutrinos travelling greater distances will exhibit greater depletion from oscillation. Moreover, the oscillation probability varies smoothly over increasing distance. Hence, tests of the angular variation of the muon rate are the best to determine whether the overall deficit of muon neutrinos fits the hypothesis of oscillation as opposed to other some unaccounted-for systematic effect. In all cases, the angular (neutrino pathlength) variation of the muon rate agrees with the oscillation hypothesis.
The oscillation probability is also a function of the neutrino energy, and here again we sample a large range of values, roughly a factor of 1000 from the Sub-GeV sample to the through-going upward muons. Although estimation of the neutrino energy giving rise to a given interaction is necessary approximate, there are no inconsistencies in the behavior of the different data sets, and all the atomospheric data agree well with the oscillation hypothesis regardless of energy.
The reason neutrino oscillation is relevant to the question of neutrino mass is that massless neutrinos cannot oscillate. Put another way, observation of oscillation implies that the masses of the neutrinos involved cannot be equal to one another. Since they cannot be equal to one another, they cannot both be zero. In fact it is quite likely that if any neutrinos have non-zero mass, all of them do.
Bio
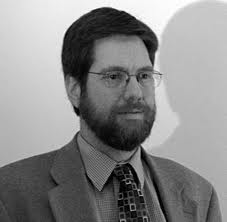
Eric D. Zimmerman is associate professor of physics at the University of Colorado, Boulder. He came to CU in 2001 as an assistant professor, and was promoted in 2008. He did undergraduate research at the MIT Plasma Fusion Center, and went on the the University of Chicago, where he received this PhD in physics in 1998. In postdoctoral work at Columbia, he began working on the neutrino, and started his involvement with the Fermilab experiments, BooNE and MiniBooNE, whose purpose is to test for neutrino mass by searching for neutrino oscillations. Neutrino mass is important because it may lead us to physics beyond the Standard Model. Masses in the range accessible to MiniBooNE expand our understanding of how the universe has evolved.
The BooNE project began in 1997. The first beam-induced neutrino events were detected in September, 2002, and the first anti-neutrino events were detected in January of 2006.
If you're good at particle physics, you'll enjoy this slide presentation Dr. Z. gave in 2007, in which a lot of snazzy neutrino physics is discussed.